Thermochemical Processing of Waste and Biomass
This review looks at the most recent advances in thermochemical processing technologies for waste and biomass conversion, focusing on pyrolysis, gasification, and combustion. It digs into current technological advancements that have increased process efficiency while reducing environmental effect, such as developments in catalyst research, reactor design, and process integration. The review evaluates the environmental benefits of various technologies, such as major pollutant reduction and carbon footprint minimization, using extensive lifetime assessments. It finishes by examining future research paths, policy ramifications, and the potential of these technologies to transform waste management and contribute to the global renewable energy mix, so encouraging a more sustainable future.
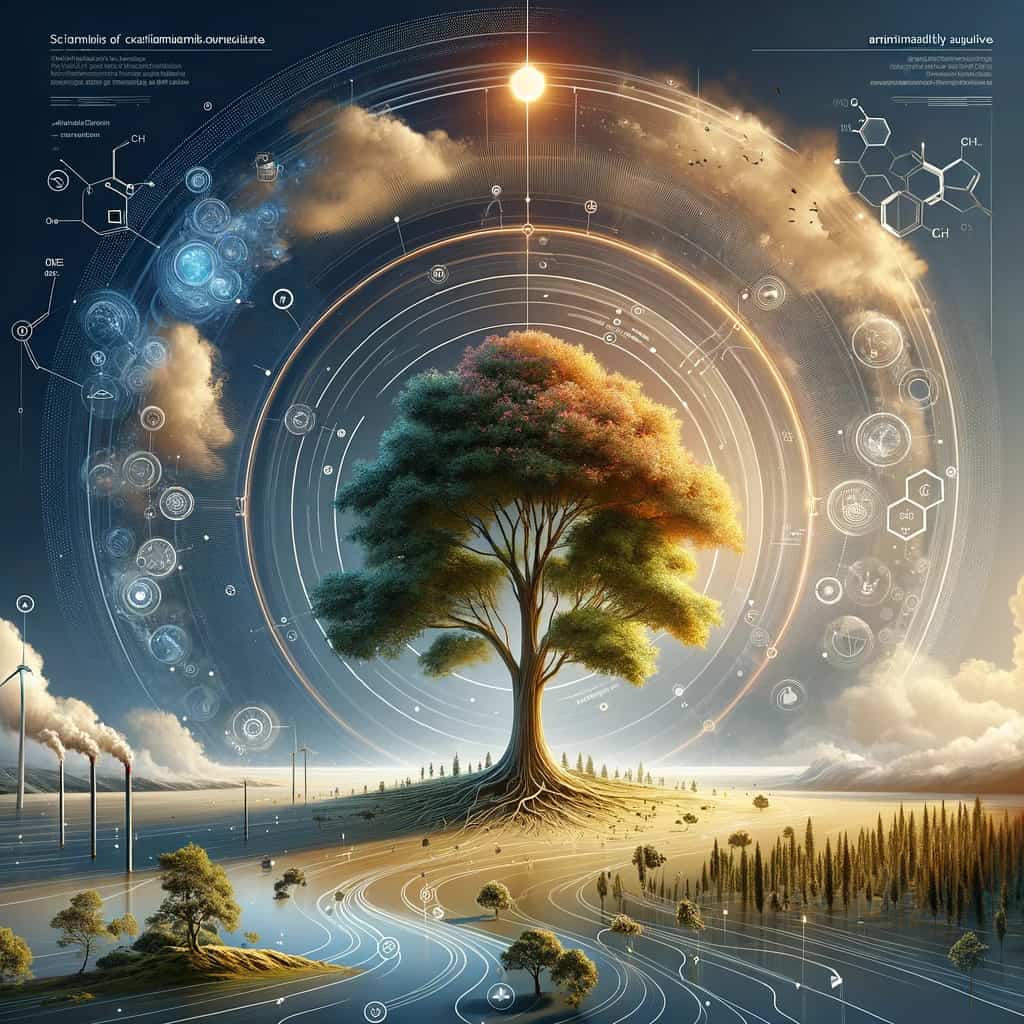
Introduction to Thermochemical Conversion
Pyrolysis, gasification, and combustion are all thermochemical conversion processes that use waste and biomass to produce energy, chemicals, and fuels. Pyrolysis is the heat degradation of organic compounds in the absence of oxygen, which produces biochar and bio-oil (Demirbas, 2009). Gasification, on the other hand, is the partial oxidation of organic molecules at high temperatures to produce syngas, a mixture of carbon monoxide, hydrogen, and other gases (Breault, 2010). Combustion is the process of burning organic materials in the presence of oxygen to produce heat energy (Breault, 2010).
Gasification is a major technology in biomass conversion because of its high efficiency and feedstock flexibility (Gao, 2023). During gasification, biomass is simultaneously pyrolyzed and gasified, with pyrolysis taking place faster than gasification (Gao, 2023). The inclusion of catalysts such as Na2CO3 or K2CO3 can improve biomass gasification, which influences coal char gasification activity (Wang et al., 2015). Co-gasification of biomass with vacuum gas oil can shed light on the kinetics of the gasification process (Al-Attas et al., 2021).
Gasification of particular materials, such as sewage sludge or cow dung, can produce hydrogen using water vapor as a gasification agent (Zhu et al., 2022). Understanding how different gasifying agents, such as air, steam, or oxygen, affect biomass gasification is critical for optimizing the process (Natarajan et al., 2022). Heating rate during pyrolysis impacts the gasification reactivity of char with CO2, affecting the overall efficiency of the process (Líu et al., 2011).
Furthermore, the composition of biomass and coal, as well as the presence of minerals, might influence gasification rates and material reactivity (Ma et al., 2014). Chemicals such as KCl can catalyze the gasification of coal char, influencing the gasification mechanism and kinetics (Qiu et al., 2022). Furthermore, the presence of iron catalysts can influence coal gasification, resulting in differences in gasification phases (Ohtsuka et al. 1987).
Thermochemical conversion methods are critical for converting trash and biomass into useful goods. Gasification, pyrolysis, and combustion offer a variety of energy generating and resource recovery options, helping to promote sustainable waste management and renewable energy production.
Technological Advances in Thermochemical Processing
Recent breakthroughs in thermochemical processing have resulted in considerable gains in efficiency and environmental effect. One important breakthrough is the construction of solar reactors for carbon dioxide splitting based on the isothermal ceria redox cycle, as proven by Hathaway et al. (2016). This design change distinguishes it from prior works and improves its efficiency in converting solar energy into useful fuels.
In the field of energy storage, Michel et al. (2014) developed a new thermochemical system that uses a hydrate salt and moist air for high-density and long-term thermal storage of solar energy. This open thermochemical system shows potential for seasonal energy storage applications, helping to promote sustainable energy solutions.
According to Rosen et al. (2010), advances in nuclear-based hydrogen production via the thermochemical copper-chlorine cycle and supercritical water reactor have showed gains in equipment scale-up and process modeling improvements. These studies highlight the possibility for integrating thermochemical cycles with nuclear reactors to efficiently use waste heat.
Catalyst development has been a major focus of recent study, with studies delving into thorough kinetic modeling and computational fluid dynamics of thermochemical processes involving solid fuels, as summarized by Choi et al. (2021). These computational approaches provide useful insights for optimizing reactions with minimal empirical factors, thereby increasing thermochemical process efficiency.
Furthermore, substantial advances in reactor design have occurred, with research focusing on minimizing exergy losses in sulfuric acid decomposition reactors for efficient hydrogen production, as mentioned by Ham et al. (2009). These advances help to improve thermochemical reactor performance by increasing exergy efficiency and decreasing entropy output.
In the field of thermochemical energy storage, numerical research have been conducted to optimize reactor designs for metal-oxide reduction processes, with a focus on the impact of starting particle size on conversion efficiency, as indicated by Muthusamy et al. (2014). These research provide important insights into improving the performance of thermochemical reactors for efficient energy storage and fuel generation.
Recent advances in thermochemical processing have centered on catalyst research, process integration, and reactor design enhancements. These innovations have not only increased efficiency but have also helped to reduce environmental impact, paving the path for more sustainable energy options.
Environmental Impact and Future Perspectives
The environmental benefits of converting trash and biomass using thermochemical processes are enormous, and they have been extensively researched. When compared to traditional approaches, thermochemical processes provide benefits in terms of lowering the carbon footprint, reducing pollutants, and enhancing overall lifespan assessments. Osman et al. (2021) found that thermochemical processes have lower environmental consequences than biological methods for the same biomass, underscoring the technologies’ sustainability.
Life cycle assessments (LCAs) are critical in determining the environmental consequences of thermochemical conversion technologies. Hu et al. (2023) investigated the entire environmental consequences of thermochemical conversion technology for algal biomass treatment and recycling using LCA approach. Similarly, Gao et al. (2021) used LCA and techno-economic analysis to evaluate the economic viability and environmental benefits of using numerous biomass feedstocks for bioenergy products via different technical pathways. These studies emphasize the significance of taking into account the complete lifecycle of waste and biomass conversion processes in order to fully comprehend their environmental impacts.
Furthermore, advances in thermochemical processes have resulted in the creation of environmentally friendly waste management and renewable energy sources. For example, Hu et al. (2023) investigated the conversion of toxic algae biomass into a CO2 reduction electrocatalyst for dual carbon use, demonstrating the possibility for new approaches to environmental concerns. Furthermore, the thermochemical conversion of sewage sludge for energy and resource recovery, as investigated by Hu et al. (2021), is a viable route for sustainable waste management methods.
In the future, research in thermochemical conversion technologies is projected to focus on improving efficiency, lowering environmental consequences, and boosting scalability of these processes. Policy implications could include promoting the use of thermochemical technologies via regulatory frameworks that promote sustainable behavior. The integration of thermochemical conversion technologies into the waste management and renewable energy sectors has the potential to have a significant global impact, providing a path to reaching larger-scale environmental sustainability goals.
In conclusion, thermochemical conversion technologies provide significant environmental benefits by lowering carbon footprints, eliminating pollutants, and enhancing lifecycle assessments when compared to traditional procedures. Future research paths and policy implications aim to improve the efficiency and sustainability of these technologies, with the potential to have a global impact on waste management and renewable energy sources.
References:
Al‐Attas, T., Lucky, R., & Hossain, M. (2021). Apparent kinetics of co‐gasification of biomass and vacuum gas oil (vgo). Chemistry – An Asian Journal, 16(5), 507-520. https://doi.org/10.1002/asia.202001271
Breault, R. (2010). Gasification processes old and new: a basic review of the major technologies. Energies, 3(2), 216-240. https://doi.org/10.3390/en3020216
Choi, C., Zhang, W., Fukumoto, K., Machida, H., & Norinaga, K. (2021). A review on detailed kinetic modeling and computational fluid dynamics of thermochemical processes of solid fuels. Energy & Fuels, 35(7), 5479-5494. https://doi.org/10.1021/acs.energyfuels.0c04052
Demirbas, A. (2009). Hydrogen-rich gases from biomass via pyrolysis and air-steam gasification. Energy Sources Part a Recovery Utilization and Environmental Effects, 31(19), 1728-1736. https://doi.org/10.1080/15567030802459693
Gao, Y. (2023). Syngas production from biomass gasification: influences of feedstock properties, reactor type, and reaction parameters. Acs Omega, 8(35), 31620-31631. https://doi.org/10.1021/acsomega.3c03050
Gao, Y., Jiang, J., Shen, Z., Xu, Z., Zeng, L., & Shao, X. (2021). Lifecycle assessment of a non-phase-transition drying pyrolysis and mass conversion technology. Energies, 14(21), 7394. https://doi.org/10.3390/en14217394
Ham, L., Gross, J., Verkooijen, A., & Kjelstrup, S. (2009). Efficient conversion of thermal energy into hydrogen: comparing two methods to reduce exergy losses in a sulfuric acid decomposition reactor. Industrial & Engineering Chemistry Research, 48(18), 8500-8507. https://doi.org/10.1021/ie801585e
Hathaway, B., Chandran, R., Gladen, A., Chase, T., & Davidson, J. (2016). Demonstration of a solar reactor for carbon dioxide splitting via the isothermal ceria redox cycle and practical implications. Energy & Fuels, 30(8), 6654-6661. https://doi.org/10.1021/acs.energyfuels.6b01265
Hu, M., Ye, Z., Zhang, H., Chen, B., Pan, Z., & Wang, J. (2021). Thermochemical conversion of sewage sludge for energy and resource recovery: technical challenges and prospects. Environmental Pollutants and Bioavailability, 33(1), 145-163. https://doi.org/10.1080/26395940.2021.1947159
Hu, X., Liu, W., Ma, L., & Yu, H. (2023). Sustainable conversion of harmful algae biomass into a co2 reduction electrocatalyst for two-fold carbon utilization. Environmental Science & Technology, 57(2), 1157-1166. https://doi.org/10.1021/acs.est.2c07145
Líu, H., Zhu, H., Yan, L., Huang, Y., Kato, S., & Kojima, T. (2011). Gasification reactivity of char with co2 at elevated temperatures: the effect of heating rate during pyrolysis. Asia-Pacific Journal of Chemical Engineering, 6(6), 905-911. https://doi.org/10.1002/apj.483
Ma, Z., Bai, J., Bai, Z., Kong, L., Guo, Z., Yan, J., … & Li, W. (2014). Mineral transformation in char and its effect on coal char gasification reactivity at high temperatures, part 2: char gasification. Energy & Fuels, 28(3), 1846-1853. https://doi.org/10.1021/ef402382m
Michel, B., Mazet, N., & Neveu, P. (2014). Experimental investigation of an innovative thermochemical process operating with a hydrate salt and moist air for thermal storage of solar energy: global performance. Applied Energy, 129, 177-186. https://doi.org/10.1016/j.apenergy.2014.04.073
Muthusamy, J., Calvet, N., & Shamim, T. (2014). Numerical investigation of a metal-oxide reduction reactor for thermochemical energy storage and solar fuel production. Energy Procedia, 61, 2054-2057. https://doi.org/10.1016/j.egypro.2014.12.074
Natarajan, A., Venugopal, D., & Lakshmanan, T. (2022). Investigation of biomass gasification simulation using air, steam and oxygen as gasifying agent. Thermal Science, 26(6 part B), 5109-5119. https://doi.org/10.2298/tsci220207092n
Ohtsuka, Y., Tamai, Y., & Tomita, A. (1987). Iron-catalyzed gasification of brown coal at low temperatures. Energy & Fuels, 1(1), 32-36. https://doi.org/10.1021/ef00001a006
Osman, A., Mehta, N., Elgarahy, A., Al-Hinai, A., Al-Muhtaseb, A., & Rooney, D. (2021). Conversion of biomass to biofuels and life cycle assessment: a review. Environmental Chemistry Letters, 19(6), 4075-4118. https://doi.org/10.1007/s10311-021-01273-0
Qiu, Q., Pan, D., Zhang, W., Zeng, F., & Liu, L. (2022). Catalytic kinetics and mechanisms of kcl with different concentrations on gasification of coal char. Processes, 10(7), 1357. https://doi.org/10.3390/pr10071357
Rosen, M., Naterer, G., Chukwu, C., Sadhankar, R., & Suppiah, S. (2010). Nuclear-based hydrogen production with a thermochemical copper-chlorine cycle and supercritical water reactor: equipment scale-up and process simulation. International Journal of Energy Research, 36(4), 456-465. https://doi.org/10.1002/er.1702
Wang, Y., Wang, Z., Huang, J., & Fang, Y. (2015). Catalytic gasification activity of na2co3 and comparison with k2co3 for a high-aluminum coal char. Energy & Fuels, 29(11), 6988-6998. https://doi.org/10.1021/acs.energyfuels.5b01537
Zhu, G., Huang, J., Wan, Z., Ling, H., & Xu, Q. (2022). Cow dung gasification process for hydrogen production using water vapor as gasification agent. Processes, 10(7), 1257. https://doi.org/10.3390/pr10071257