Deciphering the Chemical Language of the Brain
This review investigates the chemical basis of neural activity, dives into neuropharmacology and drug creation, and emphasizes cutting-edge neurochemical approaches, providing insights into the complex chemical language that orchestrates brain function.
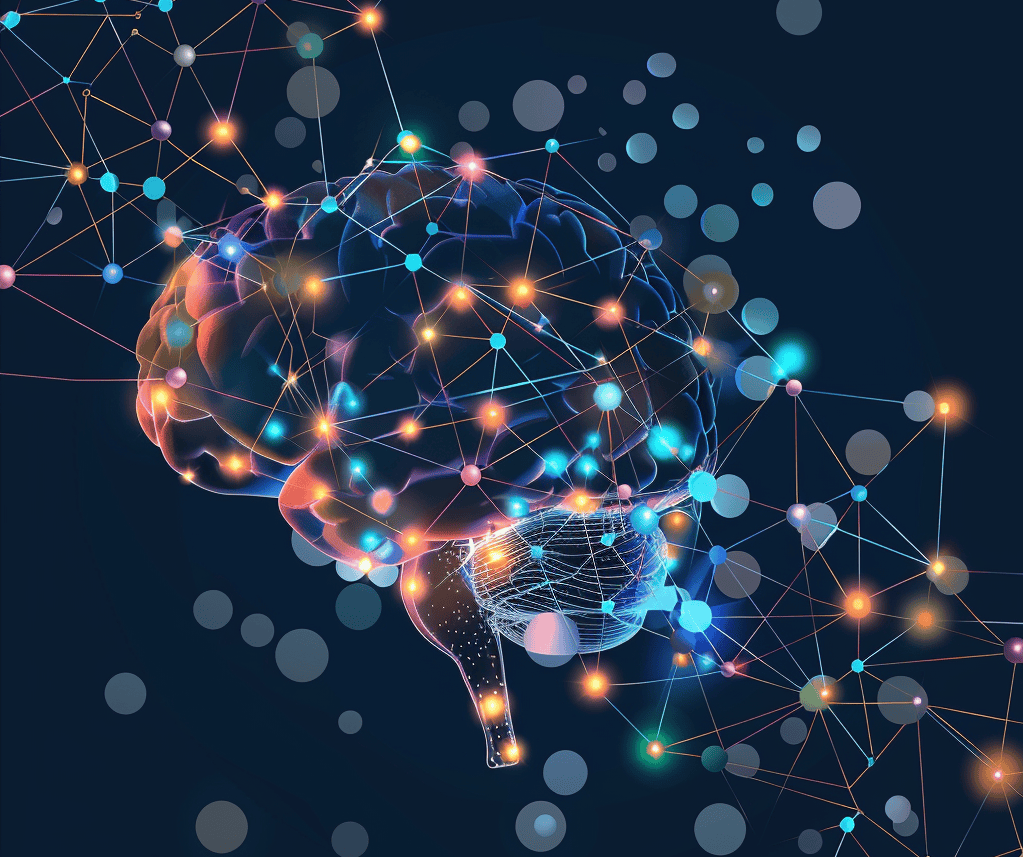
Chemical Basis of Neural Activity
Neurotransmitters facilitate neuron communication by transferring impulses between cells. Glutamate, GABA, dopamine, and serotonin are important neurotransmitters that play roles in a variety of cognitive processes. Psychiatric and neurological medications target serotonin, which modulates brain activity (El-Merahbi et al., 2015). Dopamine, on the other hand, regulates habituation and neuronal activity (Chase & Koelle, 2007). These neurotransmitters are created and released into synapses, where they exert their effects before being reabsorbed for recycling (Coleman et al., 2019).
The synthesis and release of neurotransmitters are strictly controlled processes. For example, increasing tyrosine concentrations can only increase catecholamine synthesis in neurons that are actively firing (Fernstrom & Fernstrom, 2007). Serotonin also functions as a neurotransmitter in the brain and contributes to metabolic balance (Alramadhan et al., 2012). GABA, another key neurotransmitter, regulates neuronal excitation to prevent overstimulation (Chávez et al., 2017).
Understanding neurotransmitters such as serotonin is important for a variety of applications. For example, identifying neurotransmitters is crucial to understanding brain communication (Ng et al., 2014). Furthermore, disruptions in neurotransmitter production, metabolism, and homeostasis can cause diseases affecting monoamine neurotransmitters such as serotonin and dopamine (Hyland, 2003). The intricacy of detecting neurotransmitter metabolites in peripheral fluids can lead to misdiagnoses in certain illnesses.
Neurotransmitters are essential for neuronal communication, with serotonin, dopamine, glutamate, and GABA all playing important roles in regulating numerous physiological and psychological functions. The synthesis, release, and reuptake of neurotransmitters are all closely regulated processes required for normal brain function and communication.
Neuropharmacology and Drug Design
Neuropharmacology is the study of the subtle mechanisms by which medications alter neuronal activity, particularly in the treatment of neurological illnesses. Drug design for such illnesses requires a thorough understanding of the chemical interactions between medicines and neural receptors (Gribkoff & Kaczmarek, 2017). Researchers can pave the road for more effective treatments by better understanding illness causes and how medications affect neural activity (Gribkoff & Kaczmarek, 2017).
Structure-based drug design techniques have demonstrated potential in improving medication development for neurological illnesses. By targeting specific biological medication targets with responsive medicines, researchers hope to enhance treatment outcomes for a variety of nervous system illnesses. This method requires a thorough understanding of the processes and pathology of neurological illnesses in order to build effective inhibitors of biological therapeutic targets (Aarthy et al., 2017).
Neuropharmacology is concerned with the manipulation of brain activity. Chronic antidepressant treatment, for example, has been shown to promote neurogenesis in the adult rat hippocampus, providing insight into the effects of medicines on brain function (Malberg et al., 2000). Furthermore, the use of human isolated gastrointestinal tissues has provided great insights into functional neuropharmacological studies, emphasizing the significance of translational research in understanding human neuropharmacology (Sanger et al., 2012).
Modern neuropharmacology is based on regulating neurotransmitter activity to treat a variety of brain disorders (Greenfield, 1998). Researchers hope to treat the root causes of neurological illnesses by replicating, blocking, amplifying, or decreasing the availability of certain transmitters (Greenfield, 1998). To ensure efficacy in neuropharmacology, successful drug design entails not only targeting specific receptors but also maximizing diverse pharmacological characteristics (Davis et al., 2003).
Neuropharmacology is critical in drug development for neurological illnesses because it explains how pharmaceuticals interact with neuronal receptors to influence brain function. Researchers are working to produce more effective treatments for a variety of neurological diseases by utilizing structure-based drug design approaches and understanding the principles of neurotransmitter modulation.
Advances in Neurochemical Techniques
Recent advances in neurochemical techniques have greatly aided our understanding of the nervous system’s chemistry and the consequences for brain function. Optogenetics, a technology that allows for the control of cell function via light, has played a critical role in unraveling brain networks. Pastrana (2010). Using optogenetics, researchers may precisely influence individual neuronal populations, revealing insights into the brain’s inner workings.
Neurochemical monitoring has also advanced with the introduction of in vivo neurochemical sensors. These sensors, including electrochemical and optical probes, enable real-time monitoring of neurochemical changes in living creatures (Tan et al., 2021). These discoveries have improved our understanding of neurotransmitters and neuropeptides, providing molecular insights into brain function (Zhang et al., 2008).
The combination of modern neuroimaging techniques with optogenetics has opened up new avenues for investigating brain activity and behavior. Opto-fMRI, a technology that combines optogenetics and functional magnetic resonance imaging, allows for focused manipulation of specific brain regions or cell types while also detecting global brain signaling (Beloate & Zhang, 2022). This method offers a thorough grasp of how brain activity translates into behavior.
In vivo neurochemical approaches, such as microdialysis, have been useful in understanding the neurobiological basis of reward circuits and substance-related diseases (Plaza-Zabala et al. 2012). Researchers can understand the underlying causes of seizures and drug dependence by detecting temporary neurochemical changes. This allows them to build tailored therapies.
Overall, recent advances in neurochemical techniques, including as optogenetics, in vivo neurochemical monitoring, and integrated neuroimaging approaches, have greatly enhanced our ability to research nervous system chemistry. These technologies have improved our understanding of neural networks and brain function, providing intriguing insights into neurological illnesses and potential treatments.
Reference
Aarthy, M., Panwar, U., & Selvaraj, C. (2017). Advantages of structure-based drug design approaches in neurological disorders. Current Neuropharmacology, 15(8). https://doi.org/10.2174/1570159×15666170102145257
Alramadhan, E., Hanna, M., M, H., Goldstein, T., Avila, S., & Weeks, B. (2012). Dietary and botanical anxiolytics. Medical Science Monitor, 18(4), RA40-RA48. https://doi.org/10.12659/msm.882608
Beloate, L. and Zhang, N. (2022). Connecting the dots between cell populations, whole-brain activity, and behavior. Neurophotonics, 9(03). https://doi.org/10.1117/1.nph.9.3.032208
Berger, M., Gray, J., & Roth, B. (2009). The expanded biology of serotonin. Annual Review of Medicine, 60(1), 355-366. https://doi.org/10.1146/annurev.med.60.042307.110802
Chase, D. and Koelle, M. (2007). Biogenic amine neurotransmitters in c. elegans. Wormbook. https://doi.org/10.1895/wormbook.1.132.1
Chávez, J., Hagen, J., & Kelley‐Loughnane, N. (2017). Fast and selective plasmonic serotonin detection with aptamer-gold nanoparticle conjugates. Sensors, 17(4), 681. https://doi.org/10.3390/s17040681
Coleman, J., Yang, D., Zhao, Z., Wen, P., Yoshioka, C., Tajkhorshid, E., … & Gouaux, E. (2019). Serotonin transporter–ibogaine complexes illuminate mechanisms of inhibition and transport. Nature, 569(7754), 141-145. https://doi.org/10.1038/s41586-019-1135-1
Davis, A., Teague, S., & Kleywegt, G. (2003). Application and limitations of x‐ray crystallographic data in structure‐based ligand and drug design. Angewandte Chemie, 42(24), 2718-2736. https://doi.org/10.1002/anie.200200539
El‐Merahbi, R., Löffler, M., Mayer, A., & Sumara, G. (2015). The roles of peripheral serotonin in metabolic homeostasis. Febs Letters, 589(15), 1728-1734. https://doi.org/10.1016/j.febslet.2015.05.054
Fernstrom, J. and Fernstrom, M. (2007). Tyrosine, phenylalanine, and catecholamine synthesis and function in the brain2. Journal of Nutrition, 137(6), 1539S-1547S. https://doi.org/10.1093/jn/137.6.1539s
Greenfield, S. (1998). Brain drugs of the future. BMJ, 317(7174), 1698-1701. https://doi.org/10.1136/bmj.317.7174.1698
Gribkoff, V. and Kaczmarek, L. (2017). The need for new approaches in cns drug discovery: why drugs have failed, and what can be done to improve outcomes. Neuropharmacology, 120, 11-19. https://doi.org/10.1016/j.neuropharm.2016.03.021
Hyland, K. (2003). The lumbar puncture for diagnosis of pediatric neurotransmitter diseases. Annals of Neurology, 54(S6), S13-S17. https://doi.org/10.1002/ana.10627
Malberg, J., Eisch, A., Nestler, E., & Duman, R. (2000). Chronic antidepressant treatment increases neurogenesis in adult rat hippocampus. Journal of Neuroscience, 20(24), 9104-9110. https://doi.org/10.1523/jneurosci.20-24-09104.2000
Ng, J., Heales, S., & Kurian, M. (2014). Clinical features and pharmacotherapy of childhood monoamine neurotransmitter disorders. Pediatric Drugs, 16(4), 275-291. https://doi.org/10.1007/s40272-014-0079-z
Pastrana, É. (2010). Optogenetics: controlling cell function with light. Nature Chemical Biology, 8(1), 24-25. https://doi.org/10.1038/nmeth.f.323
Plaza-Zabala, A., Maldonado, R., & Berrendero, F. (2012). The hypocretin/orexin system: implications for drug reward and relapse. Molecular Neurobiology, 45(3), 424-439. https://doi.org/10.1007/s12035-012-8255-z
Sanger, G., Broad, J., Kung, V., & Knowles, C. (2012). Translational neuropharmacology: the use of human isolated gastrointestinal tissues. British Journal of Pharmacology, 168(1), 28-43. https://doi.org/10.1111/j.1476-5381.2012.02198.x
Tan, C., Robbins, E., Wu, B., & Cui, X. (2021). Recent advances in in vivo neurochemical monitoring. Micromachines, 12(2), 208. https://doi.org/10.3390/mi12020208
Zhang, X., Rauch, A., Xiao, H., Rainer, G., & Logothetis, N. (2008). Mass spectrometry-based neurochemical analysis: perspectives for primate research. Expert Review of Proteomics, 5(5), 641-652. https://doi.org/10.1586/14789450.5.5.641