Crystal Engineering: Molecular Architectures
This review looks at crystal engineering, specifically new ways for directing molecule assembly into desired designs. It investigates applications in drug design and materials research, highlighting obstacles and future trends in the industry.
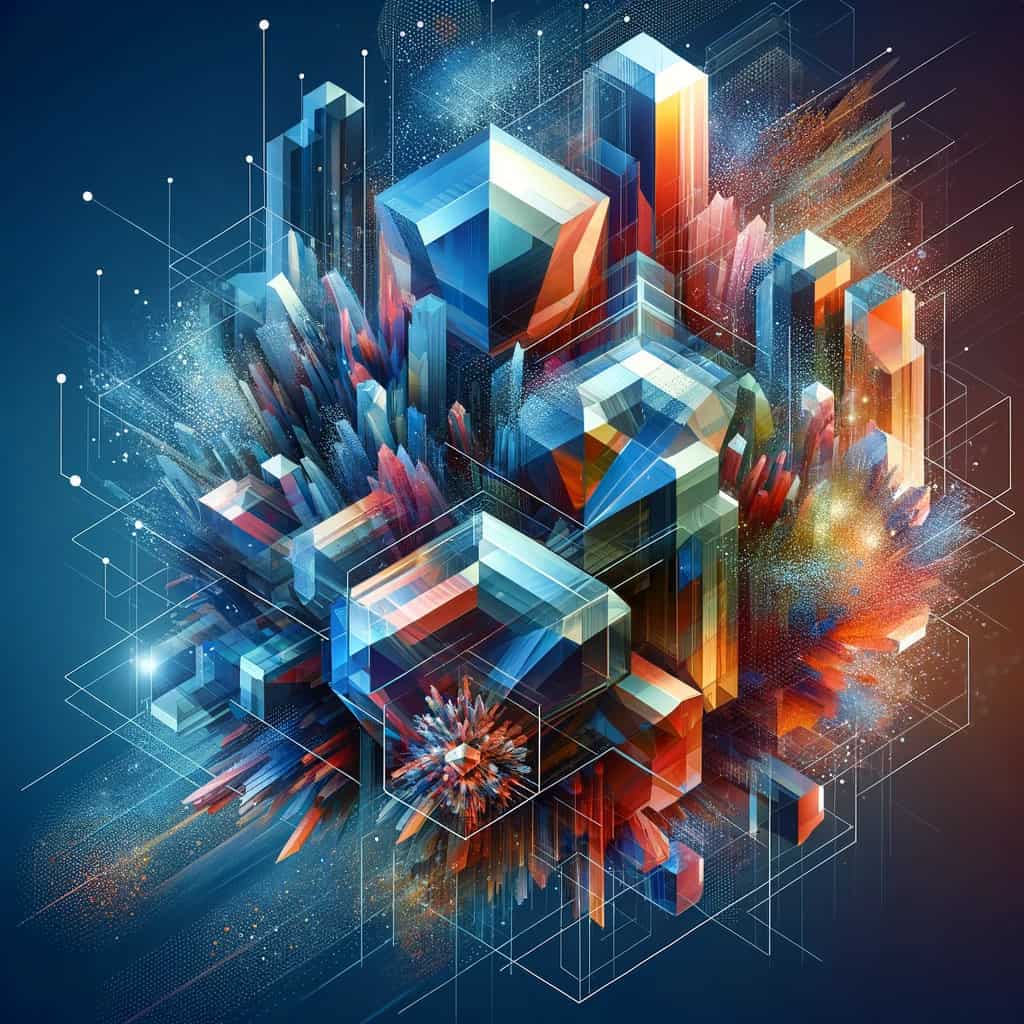
Introduction to Crystal Engineering
Crystal engineering is a multidisciplinary field that involves the deliberate design and synthesis of solid-state structures at the molecular level in order to attain desired features. Understanding intermolecular interactions and crystal packing principles is critical in this area because it allows for the creation of specialized crystal structures. Supramolecular synthons are an important topic in crystal engineering because they provide a practical foundation for crystal design and execution (Desiraju, 2013).
Crystal engineering requires a variety of interactions, including hydrogen and halogen bonding, which serve as heuristic principles for rational crystal design (Desiraju, 2002; Metrangolo et al., 2005). Furthermore, cation-π and π-π interactions are crucial in graphene-containing systems and photodimerization processes, respectively, demonstrating the varied range of interactions exploited in crystal engineering (Zhao & Zhu, 2020; Parent et al., 2014).
Crystal engineering procedures frequently involve the manipulation of crystal facets to fine-tune materials’ physicochemical properties, optimizing reactivity and selectivity (Liu et al., 2011; Wang et al., 2019). Furthermore, the usage of strained carbon nanohoops and mechanochemistry demonstrates the field’s evolution toward new types of crystal architecture and medicinal applications (Xu & Delius, 2019; Silva et al., 2020).
Crystal engineering goes beyond standard materials science and chemistry, with applications including dentistry and liquid crystal flows (Pandya & Diekwisch, 2019; Li & Walkington, 2002). Crystal engineering helps to produce adaptive soft materials and flexible devices by using molecular rotations and understanding phase transitions (Das et al., 2021).
Crystal engineering is expanding into new sectors, with an emphasis on lowering sizes and redefining crystallographic ideas. Crystal engineering, which relies on a thorough understanding of intermolecular interactions and structural features, represents a viable route for developing innovative materials with tailored functionality.
Strategies in Crystal Engineering
Crystal engineering employs a variety of ways to control the assembly of molecular components into desired crystalline networks. Key techniques include hydrogen bonding, halogen bonding, and coordination bonding. Hydrogen bonding is important in crystal engineering because it allows for directional interactions that alter crystal formations. Desiraju (1995). Halogen bonding, a strong directed interaction, has emerged as an important design factor in crystal engineering efforts, notably for coordination polymers (Dutta et al., 2022). Coordination bonding, alongside other weak interactions such as halogen bonds and π-π interactions, helps to the logical construction of crystal structures (Biradha & Santra, 2013; Aakeröy et al., 2010).
These techniques cover a wide variety of contacts, from weak interactions like halogen bonds and π-π interactions to strong hydrogen bonds and coordination complexes/polymers (Biradha and Santra, 2013). Crystal engineering uses these interactions to optimize physicochemical qualities, improve powder performance, and control crystal morphology (Biradha & Santra, 2013; Aakeröy et al., 2010). Furthermore, crystal engineering methodologies have been used to a variety of domains, including RNA crystallization, organic polymorphs for lasing media, and phytochemical alterations (Pujari et al., 2021; Tang et al., 2022; Lutfiyah et al., 2022).
Finally, crystal engineering strategies use multiple bonding interactions to tune the assembly of molecules into crystalline structures with specific qualities. These strategies have showed promise for improving material properties, refining drug formulations, and advancing sectors like as optoelectronics and medicine.
Applications and Future Directions
Crystal engineering has a wide range of applications and has a significant impact on subjects such as medication design, materials research, and nanotechnology. In drug design, crystal engineering is vital for optimizing therapeutic properties by designing pharmaceutical cocrystals, which can improve drug solubility, stability, and bioavailability. Bolla et al. (2022); Fonseca et al. (2018). Crystal engineering also has uses in nanomedicine, where created crystals are used for drug delivery systems, nanoscale imaging, and tissue engineering.
In materials science, crystal engineering plays an important role in the development of innovative materials with customized properties. Researchers can enhance material properties for specific applications by modifying crystal structures, such as optoelectronics, catalysis, and biomolecular recognition (Cavallo et al., 2016; Hui & Wong, 2020). For example, crystal shape engineering, as proven by anatase TiO2, has the potential for biomedical applications such as photodynamic treatment and drug delivery systems (Yang et al., 2015).
Crystal engineering provides significant benefits to nanotechnology, particularly in the design of nanomaterials with distinct physicochemical features. Nanoparticles created through crystal design have uses in a variety of disciplines, including medicine, electronics, and agriculture (Joudeh & Linke, 2022). The use of biosensors in cancer diagnosis and therapy, enabled by nanotechnology, demonstrates the importance of tailored crystals in improving healthcare applications (FG, 2018).
Looking ahead, there are both constraints and potential for expanding the use of tailored crystals in advanced technological applications. Crystal engineering has potential for addressing drug resistance, tissue regeneration, and environmental protection through new material design and nanotechnology applications (Bayda et al., 2019; Gatoo et al., 2014). Integrating crystal engineering principles with developing technologies such as artificial molecular machines and autonomous molecular motors creates new opportunities for nanotechnology and molecular nanotechnology (Erbaş-Çakmak et al., 2015; Wilson et al., 2016).
To summarize, the numerous applications of crystal engineering in medication design, materials science, and nanotechnology highlight its importance in improving several technical sectors. Overcoming obstacles and seizing opportunities will increase the usefulness of tailored crystals in cutting-edge applications such as healthcare, materials development, and environmental sustainability.
References:
Aakeröy, C., Champness, N., & Janiak, C. (2010). Recent advances in crystal engineering. Crystengcomm, 12(1), 22-43. https://doi.org/10.1039/b919819a
Bayda, S., Adeel, M., Tuccinardi, T., Cordani, M., & Rizzolio, F. (2019). The history of nanoscience and nanotechnology: from chemical–physical applications to nanomedicine. Molecules, 25(1), 112. https://doi.org/10.3390/molecules25010112
Biradha, K. and Santra, R. (2013). Crystal engineering of topochemical solid state reactions. Chemical Society Reviews, 42(3), 950-967. https://doi.org/10.1039/c2cs35343a
Bolla, G., Sarma, B., & Nangia, A. (2022). Crystal engineering of pharmaceutical cocrystals in the discovery and development of improved drugs. Chemical Reviews, 122(13), 11514-11603. https://doi.org/10.1021/acs.chemrev.1c00987
Cavallo, G., Metrangolo, P., Milani, R., Pilati, T., Priimägi, A., Resnati, G., … & Terraneo, G. (2016). The halogen bond. Chemical Reviews, 116(4), 2478-2601. https://doi.org/10.1021/acs.chemrev.5b00484
Das, S., Mondal, A., & Reddy, C. (2021). Harnessing molecular rotations in plastic crystals: a holistic view for crystal engineering of adaptive soft materials. Acta Crystallographica Section a Foundations and Advances, 77(a2), C1017-C1017. https://doi.org/10.1107/s0108767321086840
Desiraju, G. (1995). Supramolecular synthons in crystal engineering—a new organic synthesis. Angewandte Chemie International Edition in English, 34(21), 2311-2327. https://doi.org/10.1002/anie.199523111
Desiraju, G. (2002). Hydrogen bridges in crystal engineering: interactions without borders. Accounts of Chemical Research, 35(7), 565-573. https://doi.org/10.1021/ar010054t
Desiraju, G. (2013). Crystal engineering: from molecule to crystal. Journal of the American Chemical Society, 135(27), 9952-9967. https://doi.org/10.1021/ja403264c
Desiraju, G. (2018). Crystal engineering, crystals and crystallography. Iucrj, 5(6), 660-660. https://doi.org/10.1107/s2052252518015014
Dutta, B., Ahmed, F., & Mir, M. (2022). Halogen···halogen interactions in the context of coordination polymers: a superior design element for potential applications. Crystal Growth & Design, 22(11), 6366-6378. https://doi.org/10.1021/acs.cgd.2c00908
Erbaş-Çakmak, S., Leigh, D., McTernan, C., & Nussbaumer, A. (2015). Artificial molecular machines. Chemical Reviews, 115(18), 10081-10206. https://doi.org/10.1021/acs.chemrev.5b00146
FG, S. (2018). The use of biosensor as a new trend in cancer: bibliometric analysis from 2007 to 2017. Research & Development in Material Science, 7(5). https://doi.org/10.31031/rdms.2018.07.000675
Fonseca, J., Tenorio, J., Alvarez, N., Ellena, J., & Ayala, A. (2018). Novel solid solution of the antiretroviral drugs lamivudine and emtricitabine. Crystal Growth & Design, 18(6), 3441-3448. https://doi.org/10.1021/acs.cgd.8b00164
Gatoo, M., Naseem, S., Arfat, M., Dar, A., Qasim, K., & Zubair, S. (2014). Physicochemical properties of nanomaterials: implication in associated toxic manifestations. Biomed Research International, 2014, 1-8. https://doi.org/10.1155/2014/498420
Gavaskar, A., Rojas, D., & Videla, F. (2018). Nanotechnology: the scope and potential applications in orthopedic surgery. European Journal of Orthopaedic Surgery & Traumatology, 28(7), 1257-1260. https://doi.org/10.1007/s00590-018-2193-z
Hui, Y. and Wong, M. (2020). Application of halogen bonding to organocatalysis: a theoretical perspective. Molecules, 25(5), 1045. https://doi.org/10.3390/molecules25051045
Joudeh, N. and Linke, D. (2022). Nanoparticle classification, physicochemical properties, characterization, and applications: a comprehensive review for biologists. Journal of Nanobiotechnology, 20(1). https://doi.org/10.1186/s12951-022-01477-8
Kim, E. and Jeong, H. (2016). Current status and future direction of nanomedicine: focus on advanced biological and medical applications. Nuclear Medicine and Molecular Imaging, 51(2), 106-117. https://doi.org/10.1007/s13139-016-0435-8
Li, C. and Walkington, N. (2002). Mixed methods for the approximation of liquid crystal flows. Esaim Mathematical Modelling and Numerical Analysis, 36(2), 205-222. https://doi.org/10.1051/m2an:2002010
Liu, G., Yu, J., Lu, G., & Cheng, H. (2011). Crystal facet engineering of semiconductor photocatalysts: motivations, advances and unique properties. Chemical Communications, 47(24), 6763. https://doi.org/10.1039/c1cc10665a
Lutfiyah, D., Fitriani, L., Taher, M., & Zaini, E. (2022). Crystal engineering approach in physicochemical properties modifications of phytochemical. Science & Technology Indonesia, 7(3), 353-371. https://doi.org/10.26554/sti.2022.7.3.353-371
Metrangolo, P., Neukirch, H., Pilati, T., & Resnati, G. (2005). Halogen bonding based recognition processes: a world parallel to hydrogen bonding. Accounts of Chemical Research, 38(5), 386-395. https://doi.org/10.1021/ar0400995
Pandya, M. and Diekwisch, T. (2019). Enamel biomimetics—fiction or future of dentistry. International Journal of Oral Science, 11(1). https://doi.org/10.1038/s41368-018-0038-6
Parent, A., Ess, D., & Katzenellenbogen, J. (2014). Π–π interaction energies as determinants of the photodimerization of mono-, di-, and triazastilbenes. The Journal of Organic Chemistry, 79(12), 5448-5462. https://doi.org/10.1021/jo500457n
Pujari, N., Saundh, S., Acquah, F., Mooers, B., Ferré-D′Amaré, A., & Leung, A. (2021). Engineering crystal packing in rna structures i: past and future strategies for engineering rna packing in crystals. Crystals, 11(8), 952. https://doi.org/10.3390/cryst11080952
Silva, J., Piedade, M., André, V., Domingos, S., Martins, I., & Duarte, M. (2020). The lisbon supramolecular green story: mechanochemistry towards new forms of pharmaceuticals. Molecules, 25(11), 2705. https://doi.org/10.3390/molecules25112705
Tang, B., Tang, S., Ye, K., & Zhang, H. (2022). Emission-tunable and elastically bendable organic polymorphs for lasing media. Organic Materials, 4(04), 216-221. https://doi.org/10.1055/a-1954-3823
Wang, S., Liu, G., & Wang, L. (2019). Crystal facet engineering of photoelectrodes for photoelectrochemical water splitting. Chemical Reviews, 119(8), 5192-5247. https://doi.org/10.1021/acs.chemrev.8b00584
Wilson, M., Solà, J., Carlone, A., Goldup, S., Lebrasseur, N., & Leigh, D. (2016). An autonomous chemically fuelled small-molecule motor. Nature, 534(7606), 235-240. https://doi.org/10.1038/nature18013
Xu, Y. and Delius, M. (2019). The supramolecular chemistry of strained carbon nanohoops. Angewandte Chemie, 59(2), 559-573. https://doi.org/10.1002/anie.201906069
Yang, S., Huang, N., Jin, Y., Zhang, H., Su, Y., & Yang, H. (2015). Crystal shape engineering of anatase tio2and its biomedical applications. Crystengcomm, 17(35), 6617-6631. https://doi.org/10.1039/c5ce00804b
Zhao, G. and Zhu, H. (2020). Cation–π interactions in graphene‐containing systems for water treatment and beyond. Advanced Materials, 32(22). https://doi.org/10.1002/adma.201905756